Stars, for anyone wondering, are at the center of everything space related. And this is intended both in a figurative and quite literal way. In fact, not only do stars impact the way a group of planets, like the solar system, will form; they are also what those planets will revolve around. This is not groundbreaking information, but it is important to point out when discussing how stars are born and, eventually, die.
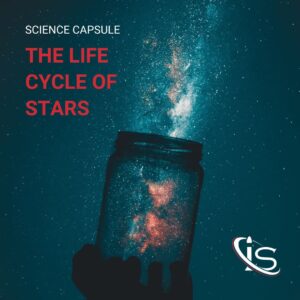
The life cycle of a star can really be broken down to three major periods. The first is when the star has not fully formed yet and is still an interstellar gas cloud. The second is the longest one, that being the time it spends on the main sequence. And, finally, the third is all the possible end states of a star. Let us break down all the defining traits for each period and how they vary for stars of different sizes.
The Interstellar Gas Cloud
The first stage for a star’s life is always the same: the interstellar gas cloud. This phenomenon, sometimes referred to as a nebula, can take on many shapes and sizes. After all, the globule of dust and gas has not turned into a star yet. In order to become a star, the gas and dust particles will have to contract under the influence of the cloud’s own gravity. To fully understand how this process works, the use of the virial theorem is necessary. This theorem, in its general definition, relates the total potential and kinetic energy of a system. However, in the field of astronomy, it is usually applied to self-gravitating objects. For an interstellar gas cloud, the theorem will look something like this:
Fortunately, we do not need to worry about how to work with the equation above to get a conceptual understanding of how a star is formed. Still, I decided to include this equation in here for any of the mathematically inclined people, like myself.
Going back to the interstellar gas cloud, once the matter inside begins condensing, it will also start heating up, leading to the formation of a protostar. This protostar, depending on how much matter it contains, will reach temperatures of up to 15 million oC. Once these temperatures are achieved, the conditions for fusing hydrogen into helium are in place.
The Main Sequence Star
As hydrogen begins fusing, the star officially enters the main sequence. This is where stars of different masses begin to differ from each other. For any star that is less than or approximately equal to the mass of the Sun, the main reaction governing the fusion of hydrogen is known as a Proton-proton Chain. On the other hand, for any star that is about 1.3 times or more the mass of the Sun, hydrogen will be primarily fused via the CNO Cycle. Now, while one type of reaction may be more prominent than the other, stars will have both occurring throughout their time on the main sequence. It just so happens that, due to these reactions being more efficient at different temperatures, individual stars will experience one more than the other. The main difference between the two is how hydrogen fuses into helium.
The Proton-proton Chain
For the Proton-proton chain, as the name suggests, two protons will fuse to produce a helium atom. In order for this to happen, the protons must have a kinetic energy that is high enough to overcome their electrostatic repulsion. This is also why this reaction is more common on certain stars, as the kinetic energy of particles inside a star is really just its temperature.
Now, the fusion of two protons into one particle does not always lead to the formation of a helium atom. In fact, most of the time, this reaction will stop at the diproton stage, as the two protons split back up. However, after enough attempts, much like a sitcom couple, the fusion of two protons will be permanent and lead to the formation of helium. Because of the tendency of protons to separate, the fusing of all hydrogen into helium in a star approximately the size of our Sun will take a very long time: about 10 billion years, in fact.
The CNO Cycle
On the other hand, we have the CNO Cycle. Once again, as the name suggests, since physicists are not very creative, this reaction involves Carbon, Nitrogen, and Oxygen. The difference here is that, unlike the Proton-proton chain, the CNO cycle is a catalytic one. This means that, instead of all the constituents being used in the reaction, the fusion of the protons will be caused by the three elements listed above acting as catalysts. Therefore, the carbon, nitrogen, and oxygen isotopes will all contribute to the fusion of four protons by being consumed at one step but will be regenerated at another. This reaction will lead to the forming of a Helium nucleus, two electron neutrinos, and two positrons.
The positrons and the electrons will annihilate each other almost immediately, releasing gamma energy. The neutrinos, meanwhile, will escape from the star while also carrying some energy. This reaction will start taking over as the dominant one at around 17×106 K, which is why it is more prominent in more massive and, therefore, hotter stars. Due to the speed at which hydrogen is consumed in this reaction, stars that use this as their predominant fusion mechanism will have much shorter lifespans on the main sequence.
The End States of Stars
After all of the star’s hydrogen has been fused, the star will move off the main sequence and into one of many possible “end states.” These can include anything from a Red Giant, to a Supernova, and even a Black Hole, so let us break them all down.
End States of Small Stars
For a star that is about as large as our Sun, or smaller, once the hydrogen has been used up, the helium core will contract even further. This will raise the temperature enough for helium to fuse into carbon. Once this happens, the outer layers of the star will start expanding and shining more dimly, creating a Red Giant. After the helium core has run out, the outer layers will detach from it and become a gaseous shell known as a Planetary Nebula. At this point, what is left of the core becomes a White Dwarf, as the star continues to cool and dim. Once the star stops giving off energy completely, it becomes a Black Dwarf and is considered dead.
End States of Massive Stars
For stars that are more massive than our Sun, the end states will vary a lot more. The first stage will look similar to that of smaller stars, as the helium core will contract and become surrounded by an expanding, cooling, gaseous shell. However, the size of said shell and core will be much larger than in a small star, leading to the formation of a Red Supergiant. Over the next millions of years, the star’s core will, eventually, become iron, with various elements forming in shells around it. The iron core, however, signals the end of any fusion process, as iron is the most stable element and would require energy absorption to fuse into something else. Therefore, the star no longer has any energy to support it against the force of gravity.
This leads to the collapse of the star’s core, an event that takes place in less than a second. Afterwards, the star undergoes an actual explosion, known as a Supernova. The resulting end states from this are two: what is left of the star after the supernova condenses into what is known as a Neutron Star. This happens for any remaining core that is between 1.5 and 3 times the mass of the Sun; what is left of the star condenses into a Black Hole. This happens for any remaining core that is more than 3 times the mass of the Sun.
The end states of stars are really fascinating, and, especially for the more massive stars, involve some very unique physical phenomena. As such, it would not do them justice to be only half explained at the end of this science capsule. Therefore, this topic will be picked back up in the near future, with another science capsule fully dedicated to it.